No living cell, to paraphrase John Donne, is an island. Even cells that qualify as complete organisms—unicellular organisms—can benefit from interactions with other cells. And mutual dependence is especially marked among the cells of multicellular organisms. These cells interact with each other and respond to environmental cues via signaling systems that are rather more sophisticated than those available to, say, free-living bacteria.
Although sophisticated signaling is essential to the survival of multicellular organisms, it also presents vulnerabilities. Even subtle signaling failures can have catastrophic consequences. The problem is, signaling mechanisms don’t stand alone, entire of themselves, any more than the cells of multicellular organisms. Signaling mechanisms link together, forming signaling chains, and in many of these chains, an extracellular event may trigger a cell surface receptor, instigating a cascade of intracellular events that may include protein phosphorylation, activation of secondary messengers, ubiquitination, or changes in gene transcription.
When signaling cascades go wrong, they may lead to malignant transformations. That is, normal cells may acquire characteristics such as limitless replicative potential, loss of anchorage dependence, resistance to apoptosis, induction of angiogenesis, and invasion of the surrounding tissues.
These modifications have a common denominator: perturbations in signaling pathways. By studying these perturbations and characterizing novel interactions, researchers hope to recognize cancer signatures, identify therapeutic targets, and develop interventions. Ultimately, at the clinical level, the manipulation of signaling pathways could put cancer into remission, prevent the emergence of resistance, and delay or even circumvent recurrence.
Overturning dogmas
“Often, dogmas that emerge in biology can inhibit the advancement of research,” says Richard A. Anderson, PhD, William R. Kellett and University Professor at the University of Wisconsin School of Medicine and Public Health. But sometimes—as Anderson has demonstrated in his own laboratory—research can overturn long-held dogmas.
An example of Anderson’s dogma-defying research concerns lipid pathways. About 20 years ago, Anderson and colleagues observed that a lipid second messenger, phosphatidylinositol 4,5-bisphosphate (PIP2), is present not just in the plasma membrane, but also in distinct subnuclear domains. These domains, called “nuclear speckles,” are in physical proximity or overlap with DNA replication, damage repair, and pre-mRNA processing foci. “This was very surprising to us,” Anderson recalls. “We wanted to understand what PIP2 was doing there.”
Present in all eukaryotic cells, including all plant, animal, and fungal cells, PIP2 has approximately 1000 effectors, including enzymes and other proteins that it regulates. “While many other secondary messengers increase in their content dramatically in response to a stimulus, PIP2 does not,” observes Anderson.
Anderson’s lab discovered that the enzymes that generate PIP2 are assembled into complexes that pass the secondary messenger to the effector proteins—much like the ball is passed in rugby, suggests Anderson. In this system, the messenger is passed very specifically back and forth between the kinases that generate it and the effectors. The nuclear pathways that involve the PIP2 effectors and their kinases are largely regulated by a wide variety of stress responses, including those for oxidative stress and DNA damage.
Anderson’s laboratory recently found, unexpectedly, that p53, one of the most comprehensively studied proteins in biology, associates with the type I phosphatidylinositol phosphate kinase and PIP2. Despite the thousands of studies on p53 and the extensive characterization of its interactome, this interaction had not been previously captured.
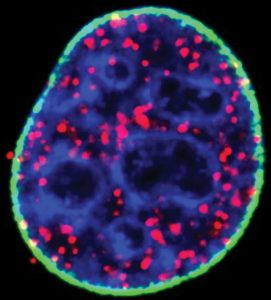
A knockdown of nuclear type I phosphatidylinositol phosphate kinase decreased nuclear PIP2 formation as well as its interaction with p53, indicating that the binding is specific and required for p53 targeting to DNA damage sites. “As we started to explore the interaction with p53,” stresses Anderson, “we discovered that the region where PIP2 binds is a key regulatory region.”
Wild-type p53 has critical functions in every cell type and is known as “the guardian of the genome.” Upon DNA damage, which happens continuously in cells, p53 induces repair pathways. But if p53 itself is damaged beyond repair, it induces cell damage. “When p53 is mutated, it becomes a different protein—from good guy to bad guy,” maintains Anderson.
It is estimated that p53 is involved in transformation and progression in close to 90% of human cancers. Mutated p53 is highly expressed in cancer cells and becomes an oncogene that can drive cancer progression rather than inducing cell death. “We discovered that p53 stability requires PIP2 binding and the phosphatidylinositol kinase,” says Anderson.
Anderson and colleagues showed that when p53 binds PIP2, it also binds a set of proteins known as small heat shock proteins, which include crystallin and hsp27. It has been known for some time that high expression of these factors in cells and tumors correlates with poor prognosis.
“We found that these proteins bind p53, and this binding is required for stability,” states Anderson. “We suspect that these small heat shock proteins are critical in defining the stability and function of mutant p53.” Targeting p53’s need for binding PIP2 and small heat shock proteins to remain stable could open vast new therapeutic possibilities.
Interrogating the kinome
“One of the implications of our work is the potential to find cancer subtype- or type-specific signatures, and possibly classify cancers based on kinases or pathways that are active,” says Maarten Altelaar, PhD, professor at the Bijvoet Center for Biomolecular Research and Utrecht Institute for Pharmaceutical Sciences, Utrecht University.
In a recent study, Altelaar and colleagues combined selective phosphopeptide enrichment with targeted mass spectrometry to develop highly specific and unbiased kinase assays. Using this approach, investigators in Altelaar’s group quantitated T-loop phosphorylation to probe activation states for 178 human kinases, which account for about one-third of the human kinome. These assays were then used in multiple cell types, primary cells, and patient-derived tissues to monitor the activation state of 73 phosphosites that belong to 63 kinase groups, creating the largest database, to date, of kinase activation sites.
“Using this strategy, we were able to see on human biopsy samples many kinases that are active,” notes Altelaar. Characterizing kinase activation states in such a system-wide manner is a promising tool for high-throughput screening and diagnostic efforts and for characterizing signaling pathways and their response to therapeutic agents. One of the challenges in therapeutics is that when the target of a signaling pathway, such as a kinase, is inhibited with a small molecule, alternative signaling pathways often become activated and provide the cell with routes for survival.
“In screening work, it would be interesting to use this technology to find combinatorial targets that prevent drug resistance from happening,” asserts Altelaar. A clinically relevant implication of this strategy is that when such rewiring is observed in cancer signaling during therapy, a patient could be taken off the drug, and later, when signaling reverts to its previous state, the drug could be reintroduced.
Screening signaling pathways to guide therapies
“One of the biggest challenges with developing inhibitors of kinases that target cell signaling pathways is that resistance develops quickly because the cells can adapt and find ways to overcome their reliance upon a certain signaling pathway,” says John Brognard, PhD, head of the Signaling Networks in Cancer Section at the National Cancer Institute. In a recent case study of a patient with a neuroendocrine tumor of unknown primary origin, Brognard and colleagues used several techniques to identify driver mutations that could be used as drug targets. Although next-generation sequencing proved disappointing, the investigators had more success with pathway analysis.
“We used a signaling screening platform that identified an activated pathway,” recounts Brognard. Pathway analysis revealed activation of the AKT pathway, and response to an AKT inhibitor was shown in vitro and in vivo.
The development of drug resistance after the accumulation of mutations is exemplified by the V600E BRAF mutation in melanoma, which is present in nearly half of patients and causes constitutive activation of the MAPK pathway, leading to enhanced cancer cell survival, apoptosis evasion, and metastasis. Even though inhibitors that target the mutation work well, resistance usually develops within six months in most patients.
“There are two approaches in the field to tackle this, and the first one relies on using combination therapies right away,” notes Brognard. For example, the combined use of BRAF and MEK inhibitors appears to shut down the pathway more potently than either of the two in isolation. “Another way the field is going—and this does not only apply to kinases and cell signaling—is the development of proteolysis-targeting chimeras,” he continues.
Developing proteolysis-targeting chimeras, known as PROTACs, involves tethering a small molecule inhibitor, such as a kinase inhibitor, to a molecule that binds an E3 ubiquitin ligase. “The activated driver enzyme would then be targeted for degradation rather than just for catalytic inhibition,” explains Brognard. In addition to their ability to degrade protein targets, PROTACs are a promising approach to help overcome or delay the development of drug resistance.
Comparing the best-laid pathways
“Our recent work on signaling didn’t originate from questions about signaling per se, but actually from questions about genetics,” says Kevin M. Haigis, PhD, associate professor of medicine at Harvard Medical School and director of cancer genetics at Beth Israel Deaconess Medical Center. One of the popular approaches to look for new therapeutic targets involves the use of synthetic lethal genetic screens. “The problem with this approach,” Haigis points out, “is that there is a tremendous amount of variability from one screen to the next.”
This variability may be explained by random noise, or it may reflect something about the biology of the system. If variability could reveal something about cancer-linked perturbations of K-RAS signaling, Haigis and colleagues would want to know what that something could be.
In a search for different contextual inputs that might alter K-RAS signaling, Haigis and colleagues performed a proteomic and phosphoproteomic analysis on mouse models of colon and pancreatic cancer, and the corresponding pre-neoplastic tissues, to explore how the tissue of origin shapes signaling. For example, over the past several years, the investigators have been applying proteomics to understand K-RAS signaling.
“The biggest obstacle,” Haigis observes, “is that the technology has rapidly outpaced the biology associated with the phosphoproteome.” Some of the experiments in Haigis’ group are powered to measure 250,000 phosphorylation sites. “Yet only a few hundred of those sites have functional annotations,” Haigis notes.
A study conducted by Haigis and colleagues identified a network of proteins that are dramatically dysregulated by oncogenic K-RAS in a tissue-specific manner, and most of the proteins in the core network were not previously connected to K-RAS signaling. The implications of the poor phosphoproteome annotation is that when K-RAS mutant and wild-type tissues are compared, the vast majority of the differences don’t have a functional readout. “This makes it very difficult to get biological insight out of that data,” admits Haigis.
Haigis and colleagues also face the challenge of determining how signaling perturbations detected in mouse tissues translate to human tissues. “The trouble in comparing human and mouse is that mice are genetically simpler and that humans are genetically more complex,” explains Haigis.
Mouse experiments can be performed in multiple replicates of genetically identical animals, reducing variability and increasing statistical power. “This makes it easier to find significant differences between K-RAS mutant and wild-type tumor samples in mice,” says Haigis. However, human experiments are not controlled in the same manner. “Every single K-RAS mutant tumor in humans is distinct from every other K-RAS mutant tumor because no two tumors are the same in terms of genetics,” Haigis points out. “This leads to a tremendous amount of variability that affects the statistical power.”
In addition to this challenge, studies are also affected by technical limitations. “We don’t have as much phosphoprotemic data from human colon cancer,” indicates Haigis, “and that limits the ability to link mouse data to human data.”