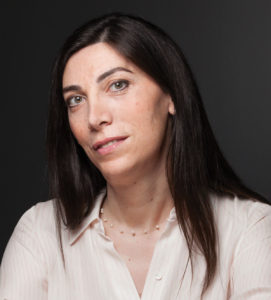
Synthetic biology has become a powerful tool to advance science, making processes more efficient, enabling completely new approaches to biology, and helping scientists find the right answers, faster.
These capabilities are having a ripple effect. They help researchers pursue avenues that had not been economically feasible or, in some cases, scientifically possible. They also help us solve some of society’s most pressing problems from infectious disease to drug development to sustainable agriculture to data storage.
Antibody development
Traditionally, to develop therapeutic antibodies, researchers immunized a rabbit or mouse to trigger an immune response, collected hits, optimized them, and ultimately humanized the winners.
Another approach is harvesting antibodies from recovered patients. During the COVID-19 pandemic, we’ve seen how patient antibodies can provide templates for further discovery and optimization. Synthetic biology allows us to rapidly synthesize the genes associated with these antibodies and create thousands of variations to develop the most precise therapeutic molecules.
Still, infectious diseases are relatively simple to address—the body’s immune system produces the original antibodies, doing much of the heavy lifting. But what about heart disease or other conditions in which there is no specific immune response and no templates to guide us?
At Twist Bioscience, we have been developing synthetic human antibody libraries, which we can produce in almost unlimited numbers. Once a laboratory identifies a protein or other potential therapeutic target, we can start with billions of fully human mutants and rapidly determine which ones will bind to that target.

One of the most important synthetic biology refinements has been automating this process: making the library, expressing the antibodies, panning them for hits, then sequencing and reformatting those hits and characterizing them through high-throughput affinity and functional testing. Based on the initial template, we can produce billions of mutants, redo the panning, and identify optimized antibodies, with a computational biology approach to confirm that these sequences exist and will, therefore, be accepted in humans.
A new vision for biopharma
This is a lot of process, but the bottom line is productivity. When we can identify therapeutic antibodies faster and more efficiently, we can take more “shots on goal,” reducing production costs and times for biologic drugs.
These advances come at an opportune time for biopharma. The more we learn about genetics and how individuals respond to specific treatments, the more it becomes apparent that a shift to therapy tailored to the individual’s specific case, known as precision medicine, could result in prognoses better than those available with currently available, widely prescribed drugs.
Today, drugs are typically prescribed based on phenotype. The best-selling drugs are those that have the highest chance of improving the lives of many individuals suffering from a common ailment, such as a type of cancer. But no two cancer cases are created equal. Instead of using the conventional approach, that is, the same drug for all patients, clinicians may employ precision medicine, which is based on next-generation sequencing (NGS) and other molecular technologies. Genomic information, for example, may be used to derive more meaning from diagnostic readings, and to find the best matches between drugs and individual patients.
The key challenge is that precision medicine requires many new therapies to benefit each patient subpopulation. Instead of having one drug that is prescribed to everyone with a reasonable chance of success, clinicians will choose from hundreds of potential drugs, each with a high probability of working if the patient’s genetics are a match. In theory, this would be a significant healthcare advancement; in practice, we must find more efficient ways to develop this new generation of personalized therapies—we need to be smarter.
Circling back to traditional antibody development approaches, we see that panning an antibody library might produce a small number of hits indicating effective antibody-target binding. However, with synthetic biology tools, researchers may find that their choices are multiplying. Instead of producing tens of clones per experiment, researchers may produce thousands, giving themselves not only additional choices, but also more flexibility.
As a result, scientists working with the same budget will have better opportunities to find the right therapeutic antibodies for smaller patient populations. This could shift the economics of drug discovery, producing more reasonably priced therapies that help more people.
There are two things that must happen. First, we need to improve our ability to identify the subpopulation of patients who will truly benefit from one drug over another, which will also help us conduct more efficient trials. Second, we must rapidly find new drugs to ensure that as many people in the population as possible will have access to effective precision medicine, and we must do so cost effectively.
That’s why efficiencies from synthetic biology and other emerging approaches are so important. As we reduce costs and speed up pipelines, we can find these precise antibodies for smaller target populations. We will still have to take a million shots on goal to find each precision drug, but development costs will be reduced, helping biopharma companies attend to these smaller populations.
In addition, we can more effectively pursue biomanufacturing, using cells to create desired compounds. These processes often focus on medicines, but the industrial applications are quite broad. Good examples include vanilla and rose oil, which are currently extracted from nature, but which can be manufactured by reengineering the metabolism of microorganisms instead. The important thing is we can use biological engineering, instead of the chemical manipulation of oil and coal feedstocks, to sustainably produce a wide variety of chemicals at scale.
The problem with plastics
From an environmental standpoint, one of the greatest disappointments over the past 20 years has been our inability to truly recycle plastics. We pay lip service to recycling and sustainability, and we praise ourselves for dumping plastic waste into ubiquitous blue containers. But we know there’s a gap between our rhetoric and the reality of waste management.
Plastics are given a number rating, 1 to 7, for their recyclability. Most people don’t pay much attention to these numbers, but they are quite important. Any plastic below a four is basically not recyclable. We may put them into the recycling bin, but they still end up in the landfill.
And even the plastics that are more readily recyclable can go through the process only once or twice. Ultimately, it’s cheaper for companies to make new plastics from scratch, using processes that require significant amounts of oil.
A couple of years ago, researchers from the United States and the United Kingdom developed a plastic-eating enzyme, called PETase, which can dissolve most plastics.1 While PETase won’t be suitable on industrial scales, it does point to a future where we can reduce plastic waste to its component parts and achieve actual recycling.
Synthetic biology offers ways to accelerate this process. As with antibodies, customizable clonal genes can help laboratories rapidly and inexpensively test thousands of enzyme variants to find the ones that most effectively break down plastics.
Made of oil
Besides paying scant attention to plastics recycling, most of us give little thought to the food supply chain, and how heavily it depends on chemical fertilizers derived from oil. It’s not farfetched to say that we are, to some degree, made of oil.
Again, we don’t have to rely on traditional oil-based approaches. Engineered bacteria can supply the nitrogen that plants need to thrive. Some research has suggested that we could create symbiotic plant/bacteria relationships, arranging for plants to request nitrogen and for bacteria to supply it.2
We can also make crop plants that are more nutritionally robust, preventing blindness and neurological disabilities in millions of children around the world. In a similar stroke, crops can be made more pest resistant, in effect reducing crop loss, land use, and soil erosion, as well as reducing reliance on environmentally problematic pesticides.
Even here, synthetic biology may hold the key to sustainability, providing the necessary tools to reduce agriculture’s reliance on chemicals, enhance soil quality, and improve global nutrition, while providing great economic benefit for farmers.
Data storage and computing
Storing data in DNA is not purely a synthetic biology application, but it captures the imagination and presents a tremendous opportunity to enhance sustainability.
Microsoft and other computing companies are based in the northwest because data storage uses a lot of energy, and Washington State has abundant, inexpensive hydroelectric power. On the other hand, DNA, the world’s most ancient data storage mechanism, can store data without using a great deal of energy. Instead of copying tape-based data archives once every 5–10 years, data repositories can store data indefinitely in test tubes of DNA.
That’s one application. Another is computing, which could end up being even more important. Although we are only at the earliest stages of this journey, we can already anticipate useful applications. Consider the simple act of copying data. Replicating a single hard drive is easy and takes only a few minutes. However, making a million copies is a much more intensive process.
But biology offers potential solutions. The enzyme polymerase can make a million DNA copies in an hour. Down the road, we will be able to use genetic material for even more complex computing tasks.
I’m excited about synthetic biology’s future because I believe it will increase efficiencies in many industries. In turn, we will reduce costs and increase our ability to develop solutions. We can do more with less and do it faster.
References
1. Austin HP, Allen MD, Donohoe BS, et al. Characterization and engineering of a plastic-degrading aromatic polyesterase. Proc. Natl. Acad. Sci. USA 2018; 115(19): E4350–E4357. DOI: 10.1073/pnas.1718804115.
2. Geddes BA, Paramasivan P, Joffrin A, et al. Engineering transkingdom signalling in plants to control gene expression in rhizosphere bacteria. Nat. Commun. 2019; 10: 3430. DOI: 10.1038/s41467-019-10882-x.
Emily Leproust, PhD is co-founder and CEO at Twist Bioscience.
To help us preview the future, we asked opinion leaders, all from outstanding technology companies, to discuss a range of new initiatives. The full list of articles is below.
Leroy Hood: Reflections on a Legendary Career
Uncharted Territory: Top Challenges Facing Gene Therapy Development
Envisioning Future Trends in Regenerative Medicine
Engineering Biology—Accelerating Transition
Bioprocessing in a Post-COVID-19 World
Sustainability and the Synthetic Biology Revolution
Sowing the Seeds of Agricultural Biotechnology
Neuroscience Widens Its Investigations of Disease Mechanisms