The extracellular matrix (ECM) is a three-dimensional (3D) network of macromolecules—such as collagen, enzymes, and glycoproteins—that is essential for spatial orientation, interaction, and signaling between surrounding cells. When ECM is engineered, the idea is to mimic natural tissue environments and gain insights into matrix-driven cellular regulation and, further, to develop replacements for damaged organs.
Imitation ECM comes to life through 3D bioprinting. An extension of traditional 3D printing, 3D bioprinting is an additive manufacturing technology that deposits biomaterials—cells, growth factors, and crosslinkable scaffold components—in arrangements favorable to the formation of tissue-like structures. 3D bioprinting is emerging as a powerful tool for regenerative medicine because it is capable of marrying optimal physical and biochemical properties for cell adhesion, migration proliferation, and differentiation.
Insight into vascular disease via 3D-printed blood vessels
3D-bioprinted models occupy a unique niche in the research community, offering tightly controlled integration of supporting matrix with relevant cell types. “If [we wanted] to create a synthetic blood vessel, we would seed endothelial cells on one of the highly biocompatible bioinks, derived from gelatin, fibrin, alginate, or other natural materials,” says Akhilesh K. Gaharwar, PhD, an associate professor of biomedical engineering at Texas A&M University.
Gaharwar’s close collaborator, Abhishek Jain, PhD, an assistant professor of biomedical engineering at Texas A&M, reinforces the point: “3D bioprinting could precisely reproduce the vascular anatomy of a patient, including personalized geometries from CT-scans. By layering a polymeric matrix and cellular communities, we incrementally increase complexity of the system, causing cells to align, interact, and respond in a physiologically, relevant manner.”
Gaharwar and Jain believe that modeling the complex vascular system could lead to better treatments of vascular disease. Accounting for over 17 million deaths per year, vascular disease is expected to reach epidemic proportions in the near future.
A complex vascular system is difficult to reproduce in vitro because it consists of a multilayered architecture of cells. This architecture is not only in keeping with basic vascular structures (vessels composed of inner and outer layers of cells), it also supports the intricate interactions that occur between vascular cells and the surrounding microenvironment.
“3D bioprinting is capable of fabricating a model that can replicate mechanical properties, such as relaxation and contraction of the walls, as well as physiological properties, such as endothelial activation, clot formation, and other important hallmarks of disease,” adds Jain. “Our laboratories are working on a new family of bioinks that retains high shape fidelity post extrusion and mechanical stability after crosslinking.”
The crosslinking results in a hydrogel, and the hydrogel is combined with disc-shaped nanosilicate nanoparticles, which add strength to the printed construct and electrostatically bind to charged polymers. Incorporation of nanosilicates into hydrogels remarkably improves the flow properties of precursor solutions of the printed product. Consequently, crosslinking printed precursors with UV light produces a uniquely strong but elastic matrix, suitable for grafting procedures.1
“Our goal is to accelerate drug discovery by creating a fully functional blood vessel with primary human vascular cells,” declares Gaharwar. “[We hope to] explore intercellular communication during disease processes at an unprecedented level.”
Tiny building blocks assembled into complex biological structures
Building blocks for bioengineered tissues are being developed at the University of Illinois at Chicago, in a laboratory led by Eben Alsberg, PhD, Richard and Loan Hill Professor of Bioengineering and Orthopedics, in collaboration with Oju Jeon, PhD, research professor of bioengineering. The building blocks are microscale hydrogels, or microgels, and they can incorporate different types of cells and/or bioactive factors. They are designed to enable LEGO-type bottom-up assembly of complex structures.2
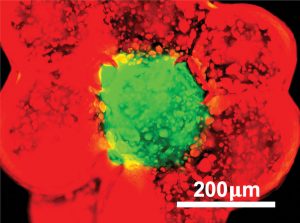
To evolve the desired architecture, microgels are printed in predefined locations into a gelatin slurry bath. The gelatin slurry helps to stabilize the embedded microgel positions. Next, the microgels are crosslinked together using light. When the slurry is melted away, the photocrosslinked microgel construct remains intact; that is, it preserves its original printed geometry. The slurry bath printing technique, which is called freeform reversible embedding of suspended hydrogels (FRESH), was developed by Adam W. Feinberg, PhD, professor of materials science and engineering and biomedical engineering at Carnegie Mellon University.
“We employed FRESH to support the assembly of ionically crosslinked alginate microgels that are additionally photocrosslinked after forming desired architectures,” explains Alsberg. “We demonstrated that the microgels, seeded with stem cells, could be frozen for long-term storage and then thawed at a later time while maintaining cell viability.
“Cryopreserved microgels could be delivered to the operating room, briefly thawed, and then printed on the spot,” he continues. “Over time, the microgel material would degrade as newly developed tissue filled the injured area.”
The microgel bioink plug-and-play characteristics are particularly advantageous for generation of complex structures, such as blood vessels or bone–cartilage interfaces. The team is making further advances in purely cellular printing, hoping to partially recreate cellular self-assembly processes present during development and healing.
“This would be advantageous, for example, in cartilage repair applications where cells are known to naturally aggregate during early chondrogenesis,” suggests Alsberg. “Cellular condensation plays a critical role in creating a suitable microenvironment for mesenchymal stem cell chondrogenic differentiation. We believe that removing physical biomaterial barriers between cells in a 3D-bioprinted construct is a whole new paradigm in the field.”3
Flash-frozen scaffolds create a conducive environment for live cells
Physical, chemical, and biological microenvironments have a direct effect on cell behavior. “We postulated that mechanical and spatial properties of 3D-printed scaffolds could significantly influence cell aggregation or morphology,“ says Stephen J. Florczyk, PhD, assistant professor of materials science and engineering at the University of Central Florida. “3D-printing processes generate fairly smooth surfaces.” These surfaces are sufficiently flat—approximately 2D at the cellular level—that they instigate distinct cellular behaviors. According to Florczyk, cells distribute themselves over flat surfaces completely differently than they do over porous, 3D surfaces.
Florczyk and colleagues noticed that in commercial 3D-printed polycaprolactone matrices, the only pores are macropores that lack the fine structural features that are needed to support matrix–cell interactions. The team set out to recreate porosity in artificial scaffolds by creatively combining two well-known techniques: FRESH (described earlier) and freeze casting.
Freeze casting, also known as freeze drying or ice templating, is still emerging in bioprinting, but it is an established method in industry, where it is used to fabricate porous materials. Freeze casting forms ice crystals within a molded material. Sublimation of ice with freeze drying leaves pores roughly the size of former ice crystals.
Various methods have been developed to exert control over the final micro- and macrostructures of freeze-cast scaffolds. Exploiting their knowledge of these methods, Florczyk and colleagues developed the Freeze-FRESH (FF) technique, where ECM scaffolds are printed in a gelatin slurry and frozen to form pores within printed struts.
The Florczyk team meticulously analyzed the material properties of FF scaffolds. Then the scientists compared these properties with those of conventionally bioprinted, freeze-cast, and commercially available biomatrices. The FF scaffolds, the scientists observed, developed micro- and macropores, reaching over 10 times the strut porosity of the conventionally bioprinted alginates.
“Remarkably, the freezing process also increased resilience of the resulting FF scaffolds,” notes Florczyk. “We envision that when mixed with additives like ceramics, FF materials could be shaped into various bone structures with mechanical properties approaching those of bone.”
The team, however, is currently pursuing another application—the modeling of the tumor microenvironment. Analyzing the signals from the tumor microenvironment in a controlled manner is challenging, but essential for understanding metastasis and disease progression. The ECM permeates the tumor, providing structural integrity and biochemical signaling.
Already, the team has collected preliminary data revealing increased cell proliferation and clustering on FF matrices. The team believes that FF scaffolds could be precisely manipulated to resemble heterogenous tumor microenvironment.
“Smart” dental materials capable of diagnostics and treatment
“A large part of biomaterials industry is focused on the use of dental polymers—primarily on the fabrication of dentures,” says Praveen R. Arany, PhD, assistant professor of oral biology and biomedical engineering at the University at Buffalo. “Dentistry has always been on the cutting edge of clinical usage of synthetic materials. Moreover, chair-side dentistry has really matured, allowing for custom fabrication of polymer-based restorations within just a few hours.”
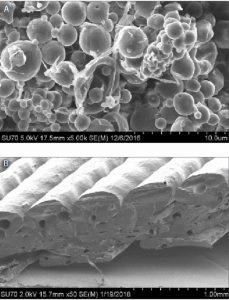
According to Arany, in the near future, subtractive (or milling) 3D techniques of creating prostheses will likely be further enhanced by additive technologies, such as printed dentures. A unique advantage of additive techniques is ability to functionalize printed material. Where tissue bioprinting incorporates cells into the matrix material, printing of dental appliances could incorporate chemicals (colors) or medicines (antibiotics and biologicals).
Arany’s lab has explored the possibility of fabricating 3D-printed dentures that encapsulate an antifungal agent, amphotericin B. Dentures-associated Candida infections affect over 65% of the denture-wearing population.4 Colonization of dentures by fungal and bacterial biofilms is notoriously hard to remove, as the organisms embed into the outer layer of polymethyl methacrylate (PMMA) material.
“We chose to emulate a well-known paradigm of drug-eluting stents,” recalls Arany. “Our results demonstrated that if antibiotic microspheres are incorporated into the outer layer of printed dentures, the drug is continuously released as the surface material degrades.”
To develop antibiotic microsphere technology with clinical potential, the Arany lab optimized the concentrations of microsphere-encapsulated drugs and the densities of microsphere. Antifungal dentures are the initial focus of OptiMed Technology, a biotechnology startup company spun off from Arany’s university lab and headquartered in Buffalo, NY. The OptiMed the team is already looking at various other applications.
“Saliva is rather underappreciated body fluid,” Arany points out. “As certain health problems arise, molecules linked to these diseases pass from the serum into the saliva. 3D printed dental appliances are uniquely positioned to support salivary diagnostics. Imagine if your dentures could measure glucose level and send data to the treating physician.”
In addition, sense-and-respond biomaterials could contribute to both the diagnosis and treatment of disease. For example, such materials could be designed to release medicines on demand. As infection sets in, the pH on the denture surface would change, awaking “smart” functionalized dental materials to initiate drug release. Arany envisions a selection of stimuli-responsive biological payloads in dental implants, surgical ligatures, and even dental instruments.
Acellular matrix turns into hydrogels to study disease
Decellularized tissues are making forays into a variety of health applications by providing scaffolds for tissue regeneration. Acellular grafts reduce the need for autologous tissue harvest from the patient and minimize chances of immune rejection.
When a tissue is decellularized, the cells that inhabit the ECM are cleared while the ECM itself is preserved. “Our laboratory was one of the first to report an apoptosis-based technique for cell removal,” asserts Young Hye Song, PhD, a lead scientist in the University of Florida laboratory led by Christine E. Schmidt, PhD, Pruitt Family Professor and chair of the department of biomedical engineering. Song suggests that the Schmidt laboratory’s approach removes cells more gently than does a common alternative, the use of harsh detergents. According to Song, harsh detergents are known to alter the tissue structure and could diminish regenerative potential of the grafts.
The team treats tissues with camptothecin, a common cytotoxin known to induce apoptosis in cells. Then the team clears away debris with mild hypertonic and isotonic buffers.
The Schmidt laboratory reports that it achieved significant success with the decellularization of nerve and lung tissue structures, showing preservation of collagen or glucosaminoglycan content. Song explains that the exact composition of ECM is still being explored. Consequently, ECM is quite difficult to reproduce by mixing individual components.
“In the future,” Song ventures, “detailed molecular analysis of decellularized ECM could bring us closer to creating ECM mixtures for personalized medicine applications.” The ultimate goal is to obtain an injectable solution of acellular graft tissue by digesting ECM with acid-enzyme preparations.
Graft implantation surgery is a complex procedure and requires excision of healthy tissue for optimal graft integration. Injectable formulations, on the other hand, may offer a less invasive option for bridging tissue lesions. Scanning electron microscopy revealed that under physiological conditions, ECM hydrogels form fibers of approximately 100 nm in diameter. The gel could be injected directly into living organisms to create scaffolds for tissue growth.
“Our future directions in lung research include recellularizing hydrogels with vascular and airway epithelial cells,” offers Song. “We could recreate microenvironments common to pulmonary diseases such as chronic obstructive pulmonary disease and develop effective therapies without a need for lung transplantation.”
References
1. Gold K, Gaharwar AK, Jain A. Emerging trends in multiscale modeling of vascular pathophysiology: Organ-on-a-chip and 3D printing. Biomaterials 2019; 196: 2–17.
2. Jeon O, Lee YB, Hinton TJ, Feinberg AW, Alsberg E. Cryopreserved cell-laden alginate microgel bioink for 3D bioprinting of living tissues. Mater. Today Chem. 2019; 12: 61–70.
3. Jeon O, Lee YB, Jeong H, Lee SJ, Alsberg E. Living cell-only bioink and photocurable supporting medium for printing and generation of engineered tissues with complex geometries. bioRxiv 2019.
4. Nagrath M, Sikora A, Graca J, Chinnici JL, Rahman SU, Reddy SG, Ponnusamy S, Maddi A, Arany PR. Functionalized prosthetic interfaces using 3D printing: Generating infection-neutralizing prosthesis in dentistry. Mater. Today Commun. 2018; 15: 114–119.